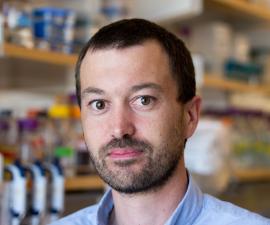
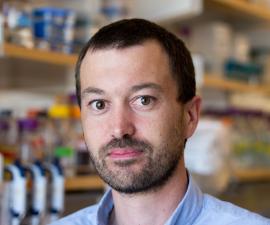
Research Expertise and Interest
biochemistry, metabolism, photosynthetic systems, Systems and Synthetic Biology, protein engineering
Research Description
David Savage is an associate professor of biochemistry, biophysics, and structural biology in the Department of Molecular and Cell Biology. The goal of the Savage Lab is to understand how protein machinery – including both enzymes and compartmentalization - facilitates the biochemistry of the cell. They are particularly interested in photosynthetic CO2 fixation, a complex set of reactions that must occur in the right place and time to capture and convert light into stored chemical energy. Photosynthesis is uniquely important for human society. It catalyzes the assimilation of inorganic carbon into the organic world and is the ultimate source of nearly all nutritional calories on Earth.
They employ several model organisms including various bacterial species, mammalian cells and plant cells. For the past several years, they have focused much of our effort on the bacterial Carbon dioxide Concentrating Mechanism (CCM), a multi-scale, multi-component system that functions to increase the rate and specificity of the critical enzyme ribulose-1,5-bisphosphate carboxylase / oxygenase (Rubisco). They use the tools of biochemistry, molecular biology, and synthetic biology to identify and characterize the key players and mechanistic principles underlying CCM function. Ultimately, they hope to develop a total cell biological understanding of how CCM function emerges from individual components and to use this understanding for the improvement of photosynthetic CO2 fixation in plants.
They are therefore also interested in the development of novel genome editing technologies for accelerating genetic experiments in plants. Given the generality of these tools, they also explore how engineered genome editors can be used to improve editing in human cells. Their specific areas of research are as follows.
Current Projects
Understanding and Improving Biological CO2 Assimilation
Cyanobacteria carry out photosynthetic carbon dioxide assimilation by controlling when and where these biochemical reactions occur. Perhaps most amazing is the use of a protein organelle, the carboxysome, to achieve improved activity of Rubisco. Rubisco is a notoriously bad enzyme, and it is thought the inside of the carboxysome provides a microenvironment within the cell of high carbon dioxide (and possibly low oxygen) as a means of improving rate and specificity. Despite numerous structural studies of carboxysome components, there are many open questions they are currently investigating related to how this massive complex self-assembles and functions in the context of the cellular milieu. For example, our systems model of the CCM (Mangan et al. 2016) suggests that pH, carbon transport, and carboxysome permeability are highly intertwined and they are interested in mechanisms that can control the permeability of the carboxysome shell. Second, work from our group has demonstrated the inherent genetic modularity of the CCM may all for it to be transferred to other organisms in order to improve their growth (Bonacci et al. 2012). They are therefore also developing tractable genetic systems to explore, in a synthetic biological context, how CCMs can be constructed de novo. They have used high-throughput screening strategies to identify unknown components of the CCM (Desmarais et al. 2019), and in a recent paper, demonstrated a complete reconstitution of the CCM by porting it from a native to a heterologous host (Flamholz et al. 2020). The latter publication is the first such successful demonstration of a fully functional, engineered CCM.
Tools for Investigating Protein Structure, Function and Engineering
Our group takes an integrative view of protein function. That is, they are interested not only in protein mechanism but also in trying to understand this mechanism in its native, cellular context. They therefore also develop genetic tools to interrogate proteins in the cell. Much of this effort has been focused on leveraging recent advances in DNA sequencing to interrogate protein function in higher throughput and also demonstrating that protein sequence topology is a critical, yet often unexplored, dimension of the sequence-function landscape (Higgins and Savage 2017). Highlights of our work include developing a novel systematic mutagenesis method (Higgins et al. 2017), a transposon-based method for rapidly constructing metabolite biosensors (Nadler et al. 2016), and development of an allosterically regulated Cas9 variant (Oakes et al. 2016). In more recent work, they have also developed a technique, called MISER, that enables the directed evolution of smaller proteins from a starting parental scaffold (Shams et al. 2021). Currently, they are developing high-throughput selective assays for some of our favorite enzymes, including Rubisco (Flamholz et al. 2020), and applying modern mutational methods and machine learning approaches to better explore sequence-function landscapes.
Genome Editing Applications
An important application of our protein engineering work is to make robust genome editing that can be delivered to the right cell at the right time. For example, the study of many model organisms, particularly plants, is constrained by our ability to quickly and easily manipulate gene sequence and gene expression. Although advances with CRISPR-Cas proteins, such as Cas9, have accelerated this progress, much remains to be done. To this end, they have developed engineered variants of Cas9 that are allosterically regulated (Oakes et al. 2016). More broadly, they have sought to optimized the entire scaffold of Cas9 using topological mutagenesis in order to improve both delivery (Oakes et al. 2019) and for effector fusions such as base editors (Huang et al. 2019). In more recent work, they have also developed a technique, called MISER, that enables the directed evolution of smaller genome editing proteins which are more suitable for viral delivery vectors (Shams et al. 2021). Current projects also include developing new genome editing tools to facilitate plant genetic experiments.
Understanding the Diversity and Functions of Protein Compartments
The carboxysome is a 250 MDa+ complex formed from thousands of proteins, yet it self-assembles in the bacterial cytosol into monodisperse particles. How does this amazing process occur? In previous work, they have used microscopy to demonstrate the importance of carboxysome partitioning during cell division and the surprising connection between this process and the bacterial cytoskeleton (Savage et al. 2010; Yokoo et al. 2015). More recently they have focused on assembly directly and have demonstrated the minimal set of proteins sufficient to produce the carboxysome in a heterologous host (Bonacci et al. 2012), and honed in on one of these proteins, CsoS2, as particularly critical to the process (Chaijarasphong et al. 2016). They are now currently building on these advances to understand how specific protein-protein interactions direct the assembly process and are attempting to elucidate the fundamental biophysical principles leading to high fidelity assembly. Finally, they now believe that the carboxysome is yet one example of myriad ways in which protein compartments can facilitate biochemical function (Nichols et al. 2017). They are thus also interested identifying novel compartments and, more broadly, understanding the functional principles of compartmentalization. An example of the former is our recent identification and characterization of a wide variety of compartments involved in sulfur metabolism (Nichols et al. 2021).
Selected Publications
Nichols RJ, LaFrance B, Phillips NR, Radford DR, Oltrogge LM, Valentin-Alvarado LE, et al. Discovery and characterization of a novel family of prokaryotic nanocompartments involved in sulfur metabolism. Elife 2021;10.
Oltrogge LM, Chaijarasphong T, Chen AW, Bolin ER, Marqusee S, Savage DF. 2020. Multivalent interactions between CsoS2 and Rubisco mediate α-carboxysome formation. Nat Struct Mol Biol 27: 281–287.
Flamholz AI, Dugan E, Blikstad C, Gleizer S, Ben-Nissan R, Amram S, et al. Functional reconstitution of a bacterial CO2 concentrating mechanism in Escherichia coli. Elife 2020;9.
Desmarais JJ, Flamholz AI, Blikstad C, Dugan EJ, Laughlin TG, Oltrogge LM, Chen AW, Wetmore K, Diamond S, Wang JY, et al. 2019. DABs are inorganic carbon pumps found throughout prokaryotic phyla. Nat Microbiol 4: 2204-2215.
Oakes BL, Fellmann C, Rishi H, Taylor KL, Ren SM, Nadler DC, Yokoo R, Arkin AP, Doudna JA, Savage DF. 2019. CRISPR-Cas9 Circular Permutants as Programmable Scaffolds for Genome Modification. Cell 176: 254–267.e16.
Hood RD, Higgins SA, Flamholz A, Nichols RJ, Savage DF. 2016. The stringent response regulates adaptation to darkness in the cyanobacterium Synechococcus elongatus. Proc Natl Acad Sci USA 113: E4867–76.
Mangan NM, Flamholz A, Hood RD, Milo R, Savage DF. 2016. pH determines the energetic efficiency of the cyanobacterial CO2 concentrating mechanism. Proc Natl Acad Sci USA 113: E5354–62.
Nadler DC, Morgan S-A, Flamholz A, Kortright KE, Savage DF. 2016. Rapid construction of metabolite biosensors using domain-insertion profiling. Nature Communications 7: 12266.
Oakes BL, Nadler DC, Flamholz A, Fellmann C, Staahl BT, Doudna JA, Savage DF. 2016. Profiling of engineering hotspots identifies an allosteric CRISPR-Cas9 switch. Nat Biotechnol 34: 646–651.
Bonacci W, Teng PK, Afonso B, Niederholtmeyer H, Grob P, Silver PA, Savage DF. 2012. Modularity of a carbon-fixing protein organelle. Proc Natl Acad Sci USA 109: 478–483.
In the News
In 10 years, CRISPR Transformed Medicine. Can It Now Help Us Deal With Climate Change?
Chan Zuckerberg Initiative Puts $11 Million Into Carbon Capture Research
Three new investigators named by Howard Hughes Medical Institute
Using two CRISPR enzymes, a COVID diagnostic in only 20 minutes
Scientists pivot to COVID-19 research, hoping for quick results to deal with pandemic
Giving Cas9 an ‘on’ switch for better control of CRISPR gene editing
Four young faculty members to receive $50,000 Sloan Research Fellowships
Four UC Berkeley faculty members have been awarded prestigious Sloan Research Fellowships, given annually by the Alfred P. Sloan Foundation to scientists, mathematicians and economists at an early stage of their careers.